When we think of rocketry, we commonly think of the impressive images and videos of spacecrafts being launched utilizing huge earth-shaking rockets, propelled through the skies by a scorching trail of exhaust and flames. These are our traditional thermochemical rocket thrusters, and since the advent of rocketry, they have been our most reliable way to get things out of our planet. They work by combusting propellant to expel gases at high velocities, generating high, almost instantaneous thrust. They boil down to simply being a really quick way to expel mass, which we know will in turn propel us in the opposite direction (thank you, Newton’s third law of motion!). However, whilst these thrusters have been (and still are!) key in our race to the stars, they do come with quite a series of complications and limitations.

To better understand these limitations, a recap of the concept of thrust and the ever-prevalent rocket equation are in order:
Back to basics:
Thrust is a fundamental concept in aerospace engineering, representing the force exerted by a propulsion system to overcome the resistance imposed by gravitational fields or forces such as drag and propel an aircraft or spacecraft forward. In our field of applications, achieving sufficient thrust is therefore crucial for achieving desired velocities and trajectories. What “sufficient” means, however, is variable, depending on whether we’re launching a satellite into orbit, manoeuvring within space, or facilitating atmospheric flight. By directly impacting the vehicle’s acceleration (and therefore also manoeuvrability and control), speed, and payload capacity, thrust is undoubtedly a key parameter in the design and performance evaluation of spacecrafts and rockets.
In analysing rocket thrusters and their viability for certain mission requirements, we find the aptly named rocket equation. In layman’s terms, it states that the faster you eject exhaust (the proper term for the mass being ejected) from a spacecraft, the less exhaust you need to execute a given manoeuvre. It’s intuitive, right? In other words, the faster our rockets exhaust speed, the faster the rocket will travel in the opposite direction for a given amount of propellant. Equivalently: the less propellant we would need to eject to raise the vehicle’s speed by a given desired amount. This conveniently boils down to conservation of momentum.
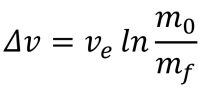
More specifically, the rocket equation relates the mass of propellant required by a rocket on a particular mission to two key velocities: the exhaust velocity (velocity at which mass is ejected from the rocket), and the mission’s delta-v (how much we need the vehicle’s velocity to change when we eject mass). For a given rocket technology (that is, a specific energy transformation and acceleration setup that produces an equally specific rocket exhaust speed), the rocket equation allows us to go from our delta-v requirement for a desired mission into the mass of propellant required to complete it. This is why delta-v is used as a metric and often taken directly as a “price tag” of a mission: the lower our required delta-v, the less propellant mass we need, or the slower we can eject it. In fact, it is often the case that the majority of a mission’s cost will boil down to the cost of transporting and ejecting propellant.
With this in mind, our recap is done! Back to the issue at hand.
Thermochemical propulsion isn’t perfect:
In traditional thermochemical rocket engines, the main limitations are their relatively low specific impulse (impulse produced per unit of propellant), limited propellant storage capacity, and high fuel consumption rates. These all drastically limit what we can feasibly do in terms of payload being carried and duration of our mission (burn times, and therefore time where thrust is generated, generally reach minutes at most for thermochemical engines!) Indeed, compared to other alternatives, these rockets achieve relatively low exhaust velocities (generally, in the order of kilometres per second) and are therefore not very efficient from a perspective of propellant mass required. This is especially noticeable if we consider that the rocket equation is exponential in nature! This relationship is why in many cases if a mission has a high delta-v requirement, the mass of propellant ends up being almost the entire mass of the spacecraft, leaving little to no room for anything else (why bother flying if we can’t carry our desired payload?)
These drawbacks demand that we explore alternative propulsion technologies. Thankfully, we have!
Introducing, electric rockets:
Colloquially also referred to as ion or ionic propulsion, this technology isn’t really new. In fact, ionic propulsion was originally thought of in the early 1900s. Sadly, due to technological constraints, it took a long time from being seen as mere science fiction to actually being implemented on a spacecraft. Though a few key scientists, such as Robert Goddard or Konstantin Tsiolkovsky did work on the technology through the beginning of this century, it didn’t really take off until the 1950s. (Do these names sound familiar? They should! The rocket equation explained earlier was independently derived by both of these scientists, after all. Turns out the founding fathers of modern rocketry and astronautics were yet again one step ahead of the curve.)


Even then, with the possibility seeming more real, the technology still faced hardship. Since the technology requires near-vacuum conditions and does not produce nearly enough thrust for lift off (more on this later), the requirements of engineers and of the technology itself resulted in thermochemical propelled rocketry being the focus of research, as it was needed to launch spacecraft. Given time, however, we successfully started launching things into space: in 1957 we saw the first successful satellite launch. Just a few years later, in 1964, we finally saw electric rocket technology in space for the first time. It took less than a decade for the aptly named SERT I (Space Electric Rocket Test I), developed by NASA, to successfully operate an ion thruster in space for just over half an hour.
Fast forwarding a bit, nowadays, electric rocket systems (notably ion thrusters and Hall effect thrusters, more on that later) are slightly more commonplace, both in real life and in science fiction (where they remain a staple; shoutout to a pretty popular fighter spacecraft named after its Twin Ion Engines in a certain franchise about war in the stars, wink wink). In real life, particularly, they still present promising alternatives to traditional rocket propulsion method.
So, how exactly do they work?
The principle behind electric rocket systems is the same as in any other rocket: eject mass in one direction, get propelled in the other. The way they achieve this, though, is completely different to thermochemical rockets. Whereas thermochemical rockets accelerate their exhaust through the combustion and expansion of their propellant, electric rocket systems utilize electromagnetic fields to accelerate and eject charged particles. More specifically, the propellant is charged or transformed into a state where it can interact with a carefully designed electromagnetic field, which promptly accelerates the propellant mass in a designated direction, out of a thruster (in occasion requiring the extra step of electrically neutralizing the particles shortly after leaving the thruster; we don’t want our charged particles to interfere with the spacecraft’s instruments, or even worse, to come shooting right back due to the attraction of opposite charges!).
This approach tends to offer a significantly higher specific impulse (in a way, a measure of how efficiently a given engine converts its propellant into thrust, as it represents the impulse generated per unit mass of propellant) compared to a thermochemical alternative. This allows us to produce thrust for considerably longer time frames by reducing propellant consumption required to reach a certain impulse. This efficiency from a mass-utilization perspective makes them ideal candidates for when missions have a long duration, as we rarely have the luxury of carrying copious amounts of fuel with us. However, these benefits are not for free. In getting a significant increase to the achieved specific impulse, we also drastically decrease the thrust that can be generated. And when I say drastically, I mean it. We’re talking millinewtons. (Picture the weight you feel holding a single sheet of paper in your hand. Yeah. Doesn’t sound too impressive when you think of ion thrusters that way). As in everything engineering, it’s a trade-off (hooray!). This inherently low thrust means we can’t yet reasonably use electric propulsion systems to achieve lift-off. However, for deep space exploration or certain station-keeping manoeuvres where drag is in essence negligible and we might need to perform small adjustments, we enter a domain where electric propulsion reigns supreme.
In the end, it’s all about understanding the mission requirements, and finding which type of propulsion is better suited for them. In fact, for different stages of one mission it is common to utilize different rocket types! How else would we get our incredible electric propulsion systems away from the atmosphere and gravity of our home planet? Graphs like the one below tend to come in handy when initially exploring our options. But wait, where’s the bubble labelled “electric” or “ionic”? Much like in thermochemical rocket engines, where solid state, liquid, or hybrid alternatives have different uses and characteristics, there is also not one universal type of electric rocket.
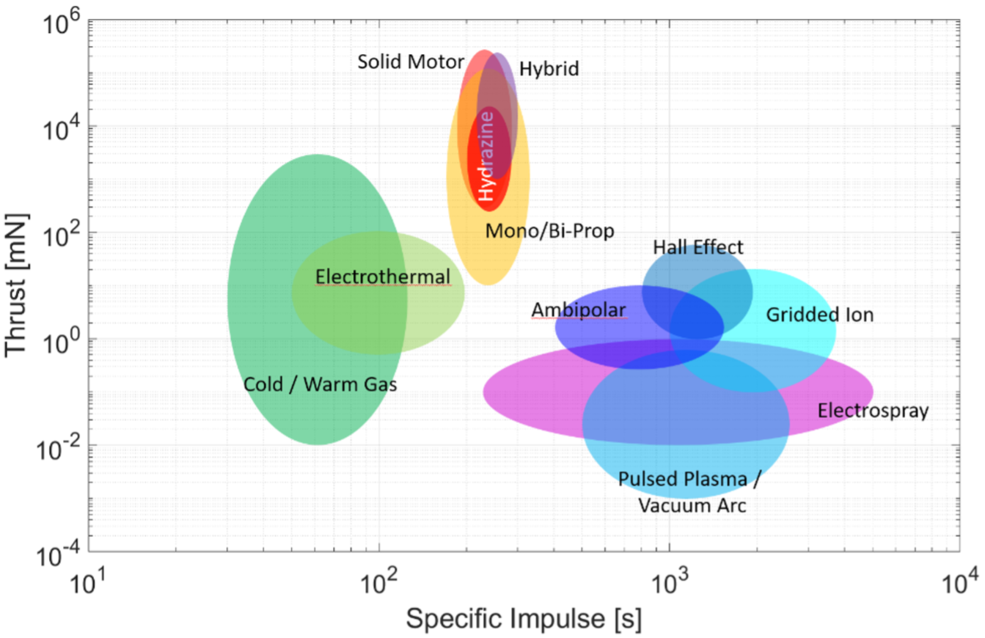
So, what are some of these alternatives, and what have they achieved, or could achieve?
Gridded Ion Systems:
First up we have the gridded ion thrusters, commonly referred to as simply ion thrusters. The idea behind these thrusters is simple (at least, as simple as things can be when it comes to rocket science!), and thus they were some of the first electric rocket engines to be built and tested. The reasoning behind their functionality is relatively straightforward and follows the basic principles outlined earlier.
If we ionize our propellant, turning it into a plasma, we can then use an electromagnetic field to eject the propellant, so long as we neutralize it down the line. In this case, neutral gasses are typically utilized. The most common is xenon, due to its non-reactive nature, and heavy atom (the more mass we eject, the more speed we pick up, remember?). However, other noble gases, or even some reactive gasses such as iodine, have seen use. Generally, in an ion thruster the gas is ionized by bombardment of electrodes emitted from a cathode. Still, other methods of ionization exist, giving subtypes of gridded ion systems, such as RF (radio frequency) ion thrusters, where the plasma is generated by exciting atoms through high energy oscillations of an electromagnetic field until they are stripped of their electrons. This takes place in the aptly named ionization chamber, our first key component of an ion thruster. The remaining electrons in either case are generally collected by a positive inner shell of the ionization chamber, with the added benefit of repelling the ion towards our next components, the grids, which are simultaneously pulling the ions towards them.
The grids, our second key component of the ion thruster, are two thin sheets of an electrically conductive material with a grid pattern (who would have thought?), placed towards the end of the ionization chamber and connected to opposite voltages of a circuit. These are the screen grid and accelerator grid, with positive and negative charges respectively. This setup generates an almost linear electromagnetic field through which our ions are accelerated and ejected at incredibly high speeds, with the repelling charge of the screen grid serving to manoeuvre the ions into the minuscule holes of the grid (this entire part is a huge oversimplification, but I invite readers to learn more about it!).
Done! Right? Nope. We’re missing something big. For our electric field to push our ions in the desired direction, the outermost grid had opposite charge to the ions. You see where I’m going with this, right? One last crucial component is needed. To ensure the ions we ejected don’t immediately get attracted right back towards the outer grid, another cathode is placed right outside the thruster. This cathode is our last key component, despite having a relatively straight forward function. It simply severes to inject electrons back into the positive ions, neutralizing them and allowing us to keep our forward momentum.
(It is worth mentioning that, as one of the oldest types of electric propulsion, it is very well developed, and many different variations exist. As such, the explanation above is by no means extensive. Entire textbooks have been written on the matter!)
So, that’s really cool and all, but have these actually served a purpose? Absolutely! Gridded ion thrusters have been used in a variety of applications and missions. But, if I had to boil it down to one, it would be NASA’s Dawn mission. Dawn was NASA’s first truly interplanetary spacecraft, launched way back in 2007, and it simply could not have achieved what it did without these thrusters. Tested some years before in the Deep Space 1 mission (launched in 1998), the NSTAR ion engine (where NSTAR stands for NASA Solar Technology Application Readiness. Yeah, on top of everything these bad boys are solar powered, adding to their sustainability) allowed Dawn to go into orbit around two different solar system bodies, giant asteroid Vesta and dwarf planet Ceres, a first for any spacecraft. Equipped with three of these 30cm diameter thrusters, Dawn kept accelerating for over 2000 days throughout its mission lifespan. Sure, the NSTAR’s thrust might have been in the order of tens of millinewtons, but over such a long period of time with virtually no resistance, this thrust stacks up, resulting in incredible speeds. This mission truly changed what we could do in outer space, and the innovation behind the thrust technology was undoubtedly an essential part of it.

Though they require complex systems and careful design, ion thrusters are tried and true. Nowadays, they have achieved internal efficiencies of over 80%, and incredibly high specific impulses well into the thousands, remaining a staple when dealing with long missions (either deep-space or station-keeping). With the technology being accepted as reliable and the enticing cost benefits of its efficiency, it is safe to say the use of these types of engines will keep increasing for the coming years.
Hall effect thrusters:
If you thought that was impressive, say hello to our most current electric propulsion workhorse: the Hall effect thruster. Again, the basic principle is to accelerate ions in order to produce thrust, but the means are different from those in a gridded ion thruster. That said, we do still tend to utilize xenon gas (if it ain’t broke, don’t fix it).
Aptly named after their underlying principle, it is the Hall effect that allows these thrusters to work. The Hall effect, in essence, is a principle of physics which describes how a potential difference is formed across a conductor in a direction orthogonal to a magnetic field and electric field, crossing said conductor. In our case, an axial electric field and radial magnetic field are generated along the axis of our thruster. As such, when electrons drift into the ionization chamber (from an external cathode and towards an internal anode), they cross the fields and start revolving around the axis of the thruster in a helicoidal manner. This is a Hall current. As our neutral propellant is injected into the same chamber, it collides with the electrons of the Hall current, currently rotating at high speeds. These collisions ionize the gas, in much the same way an electron bombardment would in a conventional gridded ion thruster. The entirety of the plasma generated is then, in essence, the conductor through which the Hall current travels, and it is therefore promptly accelerated out of the thruster by the very same electromagnetic fields that generated the ionization, through a Lorentz force.
Since the plasma holds both positive and negative charges, it does not accumulate a lot of charge to be neutralized, as in the gridded ion thruster, and any discrepancy is fixed by the fact that the plasma which might be slightly positively charged is being accelerated in the direction of the cathode releasing electrons. Convenient, right? This elegant solution makes for a significantly simpler thruster, which requires significantly less support from the rest of the spacecraft in the form of power supplies, circuits and isolation.
Compared to the gridded ion thruster, the density of the propulsive force produced by a Hall effect thruster is greater because its exhaust now contains both positive ions and electrons, the entire plasma, therefore expelling a greater amount of mass. However, their efficiency and specific impulse are slightly lesser than that achieved by their predecessor (once again, trade-offs!). It is also worth noting that we cannot yet accurately simulate and predict efficiency and thrust for these types of engines based on geometrical parameters alone. The loss of energy upon collision of electrons and neutral gas, which allows electrons to eventually reach the anode and close the circuit, does not follow our current understanding of any type of collision. In other words, with our current understanding of physics, we can’t quite understand why so much current returns to the anode. We call this enhanced anomalous transport, and its cause is currently unknown (feeling brave and studious? Here’s a cool discovery yet to be made!). Nevertheless, the physical simplicity and slightly higher thrust capability still allow Hall effect thrusters to cover a slightly different niche, and with research ongoing on the subject, more accessible and better performing Hall effect thrusters await us in the future.
That said, how have they been used? Have they actually achieved as many cool things as gridded ion thrusters? Whilst they are mostly used for station keeping, Hall effect thrusters are currently seeing flight more than ever, and there are definitely a few missions worth commenting on. We will focus on ESA’s SMART-1, which had a number of firsts for Hall effect thrusters almost 20 years ago, and NASA’s Psyche, which is following suit with a series of new firsts.

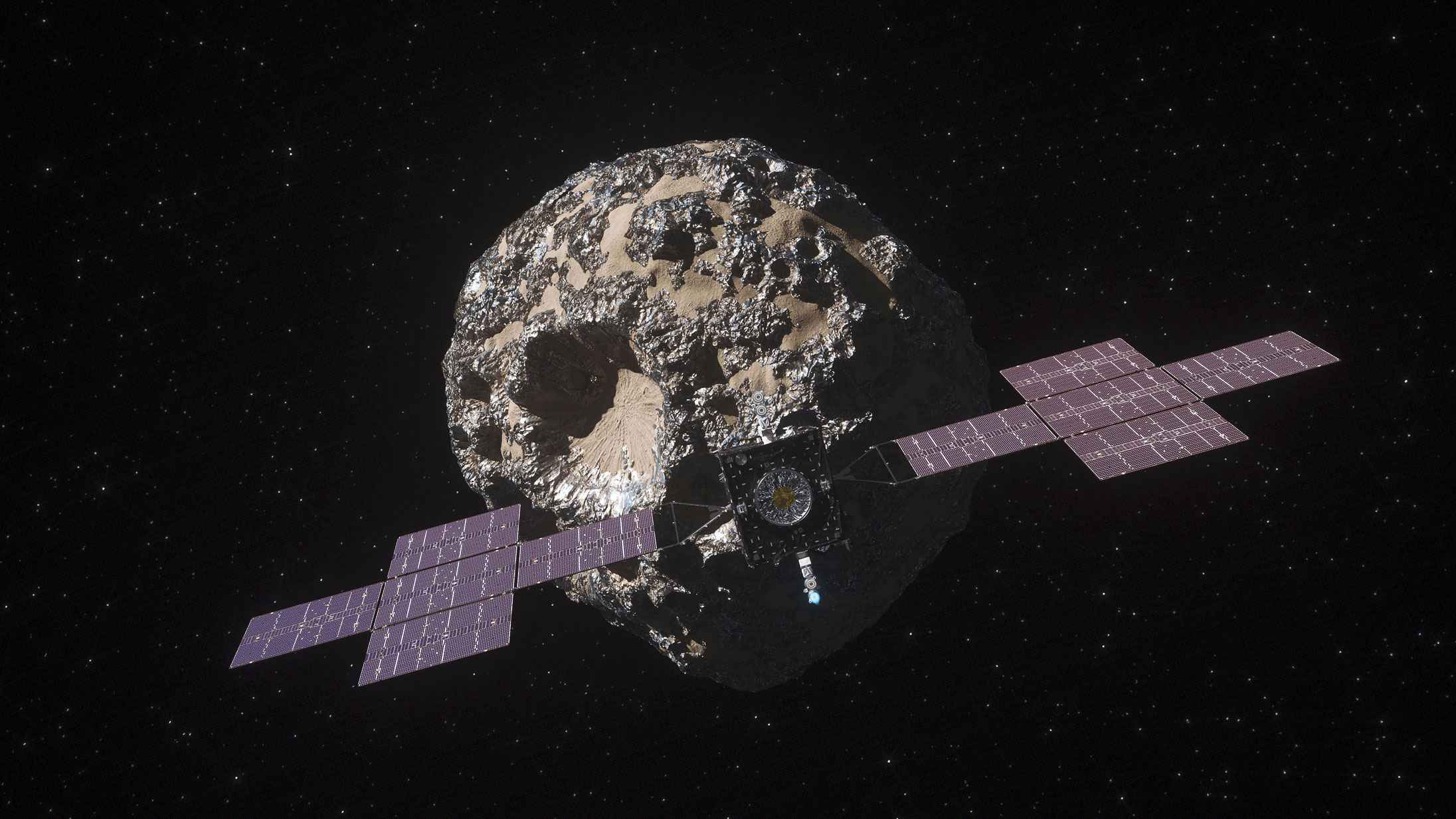
First up, SMART-1. To put it simply, this mission pioneered an incredible amount of solutions, and paved the way for missions being launched to this day. SMART-1 was ESA’s first moon mission, and in addition to investigating the moon (particularly, the chemical composition of lunar surface) it demonstrated the use of advanced ion propulsion in the form of a Hall effect thruster, also pushing forward mission control techniques for these technologies. Amongst other things, it was the first time that: electric propulsion was used to escape a geostationary transfer orbit on Earth; electric propulsion was used together with gravity-assist manoeuvres; a lunar orbiter had total mass fraction of propellant as low as 22% of its total mass; a Hall effect thruster was utilized as primary propulsion; a Hall effect thruster operated continuously for more than 240 hours in space and accumulated nearly 5000 hours of operations in space. Without a doubt, the mission was a resounding success.
A mission like this not only contributed to science in the form of all the sensors and readings it took, but it also served as a demonstration of the vast array of possibilities such thruster technologies allow. After all, it generated enough controlled thrust to successfully escape a geostationary parking orbit without auxiliary thermochemical thrusters whilst still keeping the total mass of propellant to total mass ration on a record low, setting the bar for how we can conduct similar missions. Additionally, doubts about the thruster’s reliability, fears about the inconvenience generated by low thrust (and the long manoeuvre times it tends to lead to), and exposure to varying environments were all settled by SMART-1.
Next up, Psyche. Standing on the shoulders of giants, NASA’s Psyche spacecraft is currently carrying the torch for Hall effect thrusters and electric propulsion in general. Carrying four Hall effect thrusters, each of which will operate independently and one at a time, Psyche’s task is to venture to its namesake asteroid, orbiting between Mars and Jupiter. Having launched a little under 5 months ago, the mission is still a long way from finished. The approach to the asteroid will take a long time, and initial capture is estimated around July 2029. Indeed, for over 5 years during the cruise to its destination the thrusters will be fired nearly continuously, slowly accumulating speed in the frictionless void of space. It is estimated that the craft will reach speeds of up to 200,000 kph relative to earth, easily exceeding what chemical propulsion systems are currently capable of. Once there, the spacecraft will enter start transitioning through a series of circular orbits, initially going lower in altitude and then working its way back into higher altitudes over a period of at least 26 months.
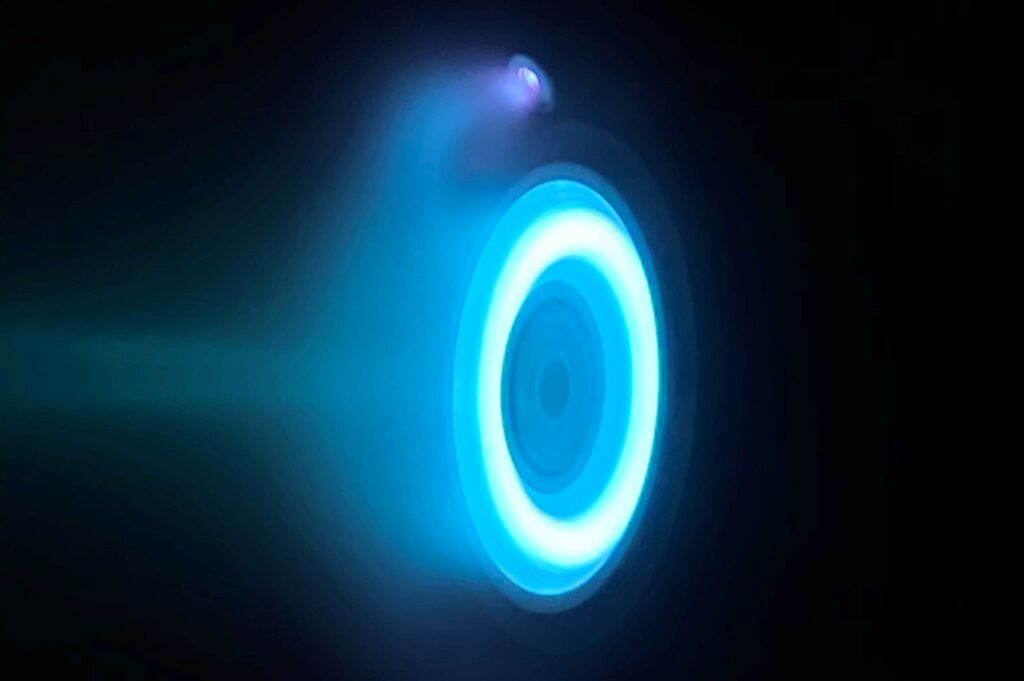
What Dawn was to Deep Space I, Psyche is to SMART-1. SMART-1 proved the technology is viable and opened up a field of possibilities for it. Now Psyche is the first spacecraft to utilize Hall effect thrusters, not only beyond earth, but in deep space. Indeed, it is the first interplanetary spacecraft operating on this kind of technology. The four Hall effect thrusters will be the primary thrust source and serve not only to reach Psyche in the first place, but to perform orbit change and orbit maintenance manoeuvres during the satellites extended stay above the asteroid. During the trajectory we will also see Psyche perform complex on-off manoeuvres to perform a gravity assist with Mars, in what is being denoted as “Mars trim maneuvers”. Additionally, we will see how the performance of the thrusters holds up when the solar array’s power output drop from 21 kW near Earth to around 3 kW during the orbits around the asteroid. With around 40% of the craft’s weight being taken up by tanks of xenon propellant, the low 22% of SMART-1 is still a truly remarkable feat, but Psyche breaks a new record in the other direction: its almost 1100 kg of xenon is more xenon used than any other space mission before it. Again, this is but a glimpse of all the things the mission strives to achieve and has achieved (if you missed it, Psyche even successfully sent a cat video back to earth from over 30,000,000 km away), and it’s just getting started. With missions like these paving the way, and thruster technologies demonstrating the possibilities, consistent interplanetary travels may not be far off in the future of our science satellites.
Magnetoplasmadynamic (MPD) thrusters:
Whew, that’s a mouthful. Looking further beyond the Hall effect thrusters which we’re still exploring now, stuck in academia and perpetual research we find the magnetoplasmadynamic thrusters. Similarly to the Hall effect thrusters, they also rely on a Lorentz force to propel the plasma out of the thruster. However, they try to avoid the issue of a higher plasma density colliding too often with the Hall current by forgoing the Hall current altogether.
In what is labelled the self-field variation of MPDs, it is the current that is radial, and it is generated between a hollow cathode and a cylindrical anode in which it is housed. This current flow creates a concentric magnetic field (this is where the self-field nomenclature comes from) within the acceleration chamber, and an outwards Lorentz force is therefore induced in the plasma while minimizing collisions with electrons. However, as we don’t have the Hall current greatly accelerating electrons and therefore increasing our thrust, we must increase the electric current flow ourselves. This leads to the first issue of the MPD. It needs to operate at very high powers to generate sufficient force to reach specific impulses where it can find its own operational niche (a thrust beyond that possible by Hall effect thrusters). And this is assuming we even generate a stable electromagnetic field and plasma in the first place.
Alternatively, we can apply an external magnetic field we generate ourselves with an external solenoid, resulting in the applied field variant of the technology. This helps us achieve our thrust even when the self-induced fields would be too weak, but again requires intensive power usage.
Whilst we’re still trying to figure this out, research even shows that, taking advantage of the charged state of the plasma, a non-tangible nozzle can be generated through the MPDs magnetic fields to increase the thrust output even further beyond the already (relative to electric propulsion) high values achieved by an MPD without this characteristic. Such a thruster can break out of the millinewtons handled by the other electric propulsion systems and into the realm of newtons, by far the highest thrust achieved by any form of electric propulsion. However, it’d still keep the relatively high specific impulse characteristic of electric propulsion. The perfect compromise. So, why don’t we use this type of propulsion?
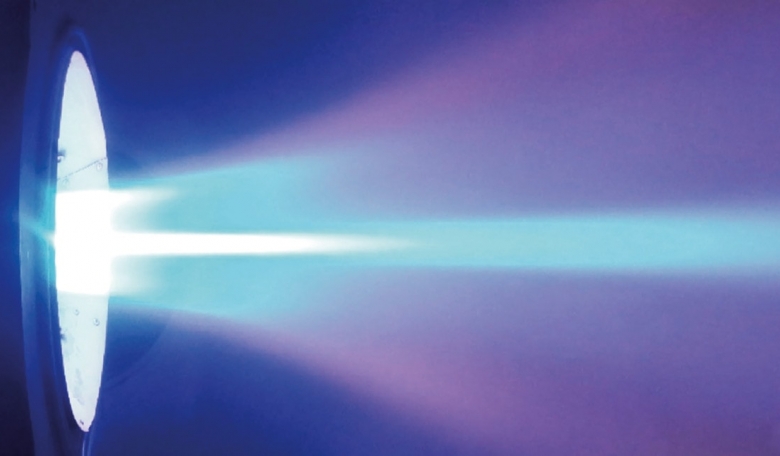
It turns out, our technology can’t quite keep up with the theory yet. To sustain such high power consumption, we’d need drastically more efficient power supplies. Indeed, nuclear power has been considered the main choice on several occasions. Even then, sustaining such high current arcs within a high pressure and high temperature plasma is complicated. Currently, the general outcome is that the electrodes corrode incredibly quickly, and the plasma is unstable and dissipates power, counteracting any possible benefit the MPDs might have over other electric propulsion systems. Nevertheless, research is still ongoing (we are even investigating this stuff at PoliMi, of course!) and promising results remind us every now and again that this technology is very much so theoretically feasible. We just need to better understand how to control its drawbacks.
So, what does the future look like?
It’s hard to say! But I for one am hopeful that electric propulsion will keep pushing the boundaries of rocketry. Projects like the DRACO mission bringing nuclear primary energy sources back into the spotlight, and materials and manufacturing technologies are providing incredible breakthroughs (shoutout to additive manufacturing) in what is possible for thruster design. Coupling this with electric propulsion proving itself time and time again, we might just be close to another breakthrough allowing us to close the gap from the theoretical to the practical. Who knows? Maybe in 20 years’ time we will have spacecrafts operating not only on solar electric propulsion, but also utilizing nuclear electric propulsion and MPDs for high thrust requirement segments of missions. ESA’s Project RocketRoll is already aiming for nuclear electric propulsion to be operational by 2035! As always, we are still pushing forward with new technologies and ideas. Each small advancement is a stepping stone towards the next, and when a small advancement takes you to the asteroid Psyche, I cannot wait to see where the path will lead with a few more stones down the line.